Ribozymes, riboswitches and beyond: regulation of gene expression without proteins
Although various functions of RNA are carried out in conjunction with proteins, some catalytic RNAs, or ribozymes, which contribute to a range of cellular processes, require little or no assistance from proteins. Furthermore, the discovery of metabolite-sensing riboswitches and other types of RNA sensors has revealed RNA-based mechanisms that cells use to regulate gene expression in response to internal and external changes. Structural studies have shown how these RNAs can carry out a range of functions. In addition, the contribution of ribozymes and riboswitches to gene expression is being revealed as far more widespread than was previously appreciated. These findings have implications for understanding how cellular functions might have evolved from RNA-based origins.
This is a preview of subscription content, access via your institution
Access options
Subscribe to this journal
Receive 12 print issues and online access
206,07 € per year
only 17,17 € per issue
Buy this article
- Purchase on SpringerLink
- Instant access to full article PDF
Prices may be subject to local taxes which are calculated during checkout
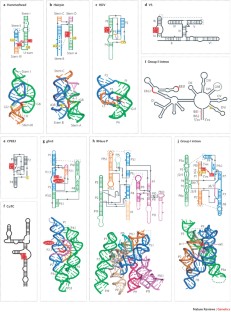


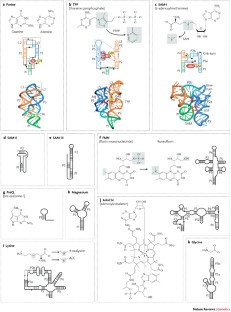
Similar content being viewed by others
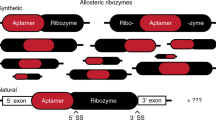
The case of the missing allosteric ribozymes
Article 25 January 2021
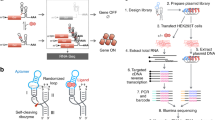
Massively parallel RNA device engineering in mammalian cells with RNA-Seq
Article Open access 23 September 2019
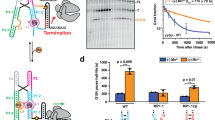
A nascent riboswitch helix orchestrates robust transcriptional regulation through signal integration
Article Open access 10 May 2024
References
- Cech, T. R., Zaug, A. J. & Grabowski, P. J. In vitro splicing of the ribosomal RNA precursor of Tetrahymena: involvement of a guanosine nucleotide in the excision of the intervening sequence. Cell27, 487–496 (1981). ArticleCASPubMedGoogle Scholar
- Guerrier-Takada, C., Gardiner, K., Marsh, T., Pace, N. & Altman, S. The RNA moiety of ribonuclease P is the catalytic subunit of the enzyme. Cell35, 849–857 (1983). ArticleCASPubMedGoogle Scholar
- Gilbert, W. Origin of life: the RNA World. Nature319, 618 (1986). ArticleGoogle Scholar
- Mironov, A. S. et al. Sensing small molecules by nascent RNA: a mechanism to control transcription in bacteria. Cell111, 747–756 (2002). References 4–6 describe the discovery of metabolite-binding riboswitches.ArticleCASPubMedGoogle Scholar
- Nahvi, A. et al. Genetic control by a metabolite binding mRNA. Chem. Biol.9, 1043–1049 (2002). ArticleCASPubMedGoogle Scholar
- Winkler, W., Nahvi, A. & Breaker, R. R. Thiamine derivatives bind messenger RNAs directly to regulate bacterial gene expression. Nature419, 952–956 (2002). ArticleCASPubMedGoogle Scholar
- Winkler, W. C., Nahvi, A., Roth, A., Collins, J. A. & Breaker, R. R. Control of gene expression by a natural metabolite-responsive ribozyme. Nature428, 281–286 (2004). This work showed that a riboswitch can function as a ribozyme.ArticleCASPubMedGoogle Scholar
- Forster, A. C. & Symons, R. H. Self-cleavage of plus and minus RNAs of a virusoid and a structural model for the active sites. Cell49, 211–220 (1987). ArticleCASPubMedGoogle Scholar
- Buzayan, J. M., Gerlach, W. L. & Bruening, G. Non-enzymatic cleavage and ligation of RNAs complementary to a plant virus satellite RNA. Nature323, 349–353 (1986). ArticleCASGoogle Scholar
- Sharmeen, L., Kuo, M. Y., Dinter-Gottlieb, G. & Taylor, J. Antigenomic RNA of human hepatitis d virus can undergo self-cleavage. J. Virol.62, 2674–2679 (1988). CASPubMedPubMed CentralGoogle Scholar
- Wu, H. N. et al. Human hepatitis δ virus RNA subfragments contain an autocleavage activity. Proc. Natl Acad. Sci USA86, 1831–1835 (1989). ArticleCASPubMedPubMed CentralGoogle Scholar
- Saville, B. J. & Collins, R. A. A site-specific self-cleavage reaction performed by a novel RNA in Neurospora mitochondria. Cell61, 685–696 (1990). ArticleCASPubMedGoogle Scholar
- Salehi-Ashtiani, K., Luptak, A., Litovchick, A. & Szostak, J. W. A genomewide search for ribozymes reveals an HDV-like sequence in the human CPEB3 gene. Science313, 1788–1792 (2006). The identification of several functional ribozymes in the human genome.ArticleCASPubMedGoogle Scholar
- Teixeira, A. et al. Autocatalytic RNA cleavage in the human β-globin pre-mRNA promotes transcription termination. Nature432, 526–530 (2004). ArticleCASPubMedGoogle Scholar
- West, S., Gromak, N. & Proudfoot, N. J. Human 5′→3′ exonuclease XRN2 promotes transcription termination at co-transcriptional cleavage sites. Nature432, 522–525 (2004). ArticleCASPubMedGoogle Scholar
- Fedor, M. J. & Williamson, J. R. The catalytic diversity of RNAs. Nature Reviews Mol. Cell Biol.6, 399–412 (2005). ArticleCASGoogle Scholar
- Kikovska, E., Svard, S. G. & Kirsebom, L. A. Eukaryotic RNase P RNA mediates cleavage in the absence of protein. Proc. Natl Acad. Sci. USA104, 2062–2067 (2007). ArticleCASPubMedPubMed CentralGoogle Scholar
- Chen, J. L. & Pace, N. R. Identification of the universally conserved core of ribonuclease P RNA. RNA3, 557–560 (1997). CASPubMedPubMed CentralGoogle Scholar
- Lindahl, L., Fretz, S., Epps, N. & Zengel, J. M. Functional equivalence of hairpins in the RNA subunits of RNase MRP and RNase P in Saccharomyces cerevisiae. RNA6, 653–658 (2000). ArticleCASPubMedPubMed CentralGoogle Scholar
- Michel, F. & Dujon, B. Conservation of RNA secondary structures in two intron families including mitochondrial-, chloroplast- and nuclear-encoded members. EMBO J.2, 33–38 (1983). ArticleCASPubMedPubMed CentralGoogle Scholar
- Michel, F., Umesono, K. & Ozeki, H. Comparative and functional anatomy of group II catalytic introns — a review. Gene82, 5–30 (1989). ArticleCASPubMedGoogle Scholar
- Pyle, A. M. & Lambowitz, A. M. in The RNA World (eds Gesteland, R. F., Cech, T. R. & Atkins, J. F.) 469–506 (Cold Spring Harbor Laboratory Press, Cold Spring Harbor, 2006). Google Scholar
- Vogel, J. & Borner, T. Lariat formation and a hydrolytic pathway in plant chloroplast group II intron splicing. EMBO J.21, 3794–3803 (2002). ArticleCASPubMedPubMed CentralGoogle Scholar
- Muller, M. W., Stocker, P., Hetzer, M. & Schweyen, R. J. Fate of the junction phosphate in alternating forward and reverse self-splicing reactions of group II intron RNA. J. Mol. Biol.222, 145–154 (1991). ArticleCASPubMedGoogle Scholar
- Nielsen, H., Westhof, E. & Johansen, S. An mRNA is capped by a 2′, 5′ lariat catalyzed by a group I-like ribozyme. Science309, 1584–1587 (2005). ArticleCASPubMedGoogle Scholar
- Altuvia, S., Kornitzer, D., Teff, D. & Oppenheim, A. B. Alternative mRNA structures of the cIII gene of bacteriophage λ determine the rate of its translation initiation. J. Mol. Biol.210, 265–280 (1989). ArticleCASPubMedGoogle Scholar
- Morita, M. T. et al. Translational induction of heat shock transcription factor sigma32: evidence for a built-in RNA thermosensor. Genes Dev.13, 655–665 (1999). ArticleCASPubMedPubMed CentralGoogle Scholar
- Johansson, J. et al. An RNA thermosensor controls expression of virulence genes in Listeria monocytogenes. Cell110, 551–561 (2002). ArticlePubMedGoogle Scholar
- Chowdhury, S., Maris, C., Allain, F. H. & Narberhaus, F. Molecular basis for temperature sensing by an RNA thermometer. EMBO J.25, 2487–2497 (2006). ArticleCASPubMedPubMed CentralGoogle Scholar
- Lybecker, M. C. & Samuels, D. S. Temperature-induced regulation of RpoS by a small RNA in Borrelia burgdorferi. Mol. Microbiol.64, 1075–1089 (2007). ArticleCASPubMedGoogle Scholar
- Shamovsky, I., Ivannikov, M., Kandel, E. S., Gershon, D. & Nudler, E. RNA-mediated response to heat shock in mammalian cells. Nature440, 556–560 (2006). ArticleCASPubMedGoogle Scholar
- Kolb, F. A. et al. Progression of a loop–loop complex to a four-way junction is crucial for the activity of a regulatory antisense RNA. EMBO J.19, 5905–5915 (2000). ArticleCASPubMedPubMed CentralGoogle Scholar
- Lease, R. A., Cusick, M. E. & Belfort, M. Riboregulation in Escherichia coli: DsrA RNA acts by RNA:RNA interactions at multiple loci. Proc. Natl Acad. Sci. USA95, 12456–12461 (1998). ArticleCASPubMedPubMed CentralGoogle Scholar
- Majdalani, N., Cunning, C., Sledjeski, D., Elliott, T. & Gottesman, S. DsrA RNA regulates translation of RpoS message by an anti-antisense mechanism, independent of its action as an antisilencer of transcription. Proc. Natl Acad. Sci. USA95, 12462–12467 (1998). ArticleCASPubMedPubMed CentralGoogle Scholar
- Altuvia, S., Weinstein-Fischer, D., Zhang, A., Postow, L. & Storz, G. A small, stable RNA induced by oxidative stress: role as a pleiotropic regulator and antimutator. Cell90, 43–53 (1997). ArticleCASPubMedGoogle Scholar
- Altuvia, S., Zhang, A., Argaman, L., Tiwari, A. & Storz, G. The Escherichia coli OxyS regulatory RNA represses fhlA translation by blocking ribosome binding. EMBO J.17, 6069–6075 (1998). ArticleCASPubMedPubMed CentralGoogle Scholar
- Zhang, A. et al. The OxyS regulatory RNA represses rpoS translation and binds the Hfq (HF-I) protein. EMBO J.17, 6061–6068 (1998). ArticleCASPubMedPubMed CentralGoogle Scholar
- Zhang, A., Wassarman, K. M., Ortega, J., Steven, A. C. & Storz, G. The Sm-like Hfq protein increases OxyS RNA interaction with target mRNAs. Mol. Cell9, 11–22 (2002). ArticlePubMedGoogle Scholar
- Grundy, F. J. & Henkin, T. M. tRNA as a positive regulator of transcription antitermination in B. subtilis. Cell74, 475–482 (1993). ArticleCASPubMedGoogle Scholar
- Grundy, F. J., Winkler, W. C. & Henkin, T. M. tRNA-mediated transcription antitermination in vitro: codon–anticodon pairing independent of the ribosome. Proc. Natl Acad. Sci. USA99, 11121–11126 (2002). ArticleCASPubMedPubMed CentralGoogle Scholar
- Gagnon, Y. et al. Clustering and co-transcription of the Bacillus subtilis genes encoding the aminoacyl-tRNA synthetases specific for glutamate and for cysteine and the first enzyme for cysteine biosynthesis. J. Biol. Chem.269, 7473–7482 (1994). CASPubMedGoogle Scholar
- Breaker, R. R. in The RNA World (eds Gesteland, R. F., Cech, T. R. & Atkins, J. F.) 89–108 (Cold Spring Harbor Laboratory Press, Cold Spring Harbor, 2006). Google Scholar
- Cheah, M. T., Wachter, A., Sudarsan, N. & Breaker, R. R. Control of alternative RNA splicing and gene expression by eukaryotic riboswitches. Nature447, 391–393 (2007). References43and44established the involvement of eukaryotic riboswitches in the control of alternative splicing.ArticleCASGoogle Scholar
- Kubodera, T. et al. Thiamine-regulated gene expression of Aspergillus oryzae thiA requires splicing of the intron containing a riboswitch-like domain in the 5′-UTR. FEBS Lett.555, 516–520 (2003). ArticleCASPubMedGoogle Scholar
- Mandal, M. & Breaker, R. R. Adenine riboswitches and gene activation by disruption of a transcription terminator. Nature Struct. Mol. Biol.11, 29–35 (2004). ArticleCASGoogle Scholar
- Corbino, K. A. et al. Evidence for a second class of S-adenosylmethionine riboswitches and other regulatory RNA motifs in α-proteobacteria. Genome Biol.6, R70 (2005). ArticleCASPubMedPubMed CentralGoogle Scholar
- Epshtein, V., Mironov, A. S. & Nudler, E. The riboswitch-mediated control of sulfur metabolism in bacteria. Proc. Natl Acad. Sci. USA100, 5052–5056 (2003). ArticleCASPubMedPubMed CentralGoogle Scholar
- Fuchs, R. T., Grundy, F. J. & Henkin, T. M. The SMK box is a new SAM-binding RNA for translational regulation of SAM synthetase. Nature Struct. Mol. Biol.13, 226–233 (2006). ArticleCASGoogle Scholar
- McDaniel, B. A., Grundy, F. J., Artsimovitch, I. & Henkin, T. M. Transcription termination control of the S box system: direct measurement of S-adenosylmethionine by the leader RNA. Proc. Natl Acad. Sci. USA100, 3083–3088 (2003). ArticleCASPubMedPubMed CentralGoogle Scholar
- Winkler, W. C., Cohen-Chalamish, S. & Breaker, R. R. An mRNA structure that controls gene expression by binding FMN. Proc. Natl Acad. Sci. USA99, 15908–15913 (2002). ArticleCASPubMedPubMed CentralGoogle Scholar
- Nou, X. & Kadner, R. J. Adenosylcobalamin inhibits ribosome binding to btuB RNA. Proc. Natl Acad. Sci. USA97, 7190–7195 (2000). ArticleCASPubMedPubMed CentralGoogle Scholar
- Winkler, W. C., Nahvi, A., Sudarsan, N., Barrick, J. E. & Breaker, R. R. An mRNA structure that controls gene expression by binding S-adenosylmethionine. Nature Struct. Biol.10, 701–707 (2003). ArticleCASPubMedGoogle Scholar
- Mandal, M. et al. A glycine-dependent riboswitch that uses cooperative binding to control gene expression. Science306, 275–279 (2004). ArticleCASPubMedGoogle Scholar
- Sudarsan, N., Wickiser, J. K., Nakamura, S., Ebert, M. S. & Breaker, R. R. An mRNA structure in bacteria that controls gene expression by binding lysine. Genes Dev.17, 2688–2697 (2003). ArticleCASPubMedPubMed CentralGoogle Scholar
- Mandal, M., Boese, B., Barrick, J. E., Winkler, W. C. & Breaker, R. R. Riboswitches control fundamental biochemical pathways in Bacillus subtilis and other bacteria. Cell113, 577–586 (2003). ArticleCASPubMedGoogle Scholar
- Roth, A. et al. A riboswitch selective for the queuosine precursor preQ1 contains an unusually small aptamer domain. Nature Struct. Mol. Biol.14, 308–317 (2007). ArticleCASGoogle Scholar
- Cromie, M. J., Shi, Y., Latifi, T. & Groisman, E. A. An RNA sensor for intracellular Mg 2+ . Cell125, 71–84 (2006). ArticleCASPubMedGoogle Scholar
- Barrick, J. E. et al. New RNA motifs suggest an expanded scope for riboswitches in bacterial genetic control. Proc. Natl Acad. Sci. USA101, 6421–6426 (2004). ArticleCASPubMedPubMed CentralGoogle Scholar
- Sudarsan, N., Cohen-Chalamish, S., Nakamura, S., Emilsson, G. M. & Breaker, R. R. Thiamine pyrophosphate riboswitches are targets for the antimicrobial compound pyrithiamine. Chem. Biol.12, 1325–1335 (2005). ArticleCASPubMedGoogle Scholar
- Blount, K. F., Wang, J. X., Lim, J., Sudarsan, N. & Breaker, R. R. Antibacterial lysine analogs that target lysine riboswitches. Nature Chem. Biol.3, 44–49 (2007). ArticleCASGoogle Scholar
- Blount, K. F. & Breaker, R. R. Riboswitches as antibacterial drug targets. Nature Biotechnol.24, 1558–1564 (2006). ArticleCASGoogle Scholar
- Batey, R. T., Gilbert, S. D. & Montange, R. K. Structure of a natural guanine-responsive riboswitch complexed with the metabolite hypoxanthine. Nature432, 411–415 (2004). References62and66describe the first three-dimensional structures of riboswitches.ArticleCASPubMedGoogle Scholar
- Edwards, T. E. & Ferre-D'Amare, A. R. Crystal structures of the thi-box riboswitch bound to thiamine pyrophosphate analogs reveal adaptive RNA–small molecule recognition. Structure14, 1459–1468 (2006). ArticleCASPubMedGoogle Scholar
- Montange, R. K. & Batey, R. T. Structure of the S-adenosylmethionine riboswitch regulatory mRNA element. Nature441, 1172–1175 (2006). ArticleCASPubMedGoogle Scholar
- Serganov, A., Polonskaia, A., Phan, A. T., Breaker, R. R. & Patel, D. J. Structural basis for gene regulation by a thiamine pyrophosphate-sensing riboswitch. Nature441, 1167–1171 (2006). ArticleCASPubMedPubMed CentralGoogle Scholar
- Serganov, A. et al. Structural basis for discriminative regulation of gene expression by adenine- and guanine-sensing mRNAs. Chem. Biol.11, 1729–1741 (2004). ArticleCASPubMedPubMed CentralGoogle Scholar
- Thore, S., Leibundgut, M. & Ban, N. Structure of the eukaryotic thiamine pyrophosphate riboswitch with its regulatory ligand. Science312, 1208–1211 (2006). ArticleCASPubMedGoogle Scholar
- Adams, P. L., Stahley, M. R., Kosek, A. B., Wang, J. & Strobel, S. A. Crystal structure of a self-splicing group I intron with both exons. Nature430, 45–50 (2004). References68, 71, 72and79provide insights into the group I intron self-splicing mechanism at the atomic level.ArticleCASPubMedGoogle Scholar
- Cochrane, J. C., Lipchock, S. V. & Strobel, S. A. Structural investigation of the GlmS ribozyme bound to its catalytic cofactor. Chem. Biol.14, 97–105 (2007). References69and75describe the crystal structures of theglmSribozyme.ArticleCASPubMedGoogle Scholar
- Ferre-D'Amare, A. R., Zhou, K. & Doudna, J. A. Crystal structure of a hepatitis δ virus ribozyme. Nature395, 567–574 (1998). ArticleCASPubMedGoogle Scholar
- Golden, B. L., Kim, H. & Chase, E. Crystal structure of a phage Twort group I ribozyme-product complex. Nature Struct. Mol. Biol.12, 82–89 (2005). ArticleCASGoogle Scholar
- Guo, F., Gooding, A. R. & Cech, T. R. Structure of the Tetrahymena ribozyme: base triple sandwich and metal ion at the active site. Mol. Cell16, 351–362 (2004). CASPubMedGoogle Scholar
- Kazantsev, A. V. et al. Crystal structure of a bacterial ribonuclease P RNA. Proc. Natl Acad. Sci. USA102, 13392–13397 (2005). ArticleCASPubMedPubMed CentralGoogle Scholar
- Ke, A., Zhou, K., Ding, F., Cate, J. H. & Doudna, J. A. A conformational switch controls hepatitis d virus ribozyme catalysis. Nature429, 201–205 (2004). ArticleCASPubMedGoogle Scholar
- Klein, D. J. & Ferre-D'Amare, A. R. Structural basis of glmS ribozyme activation by glucosamine-6-phosphate. Science313, 1752–1756 (2006). ArticleCASPubMedGoogle Scholar
- Martick, M. & Scott, W. G. Tertiary contacts distant from the active site prime a ribozyme for catalysis. Cell126, 309–320 (2006). ArticleCASPubMedPubMed CentralGoogle Scholar
- Rupert, P. B. & Ferre-D'Amare, A. R. Crystal structure of a hairpin ribozyme-inhibitor complex with implications for catalysis. Nature410, 780–786 (2001). ArticleCASPubMedGoogle Scholar
- Rupert, P. B., Massey, A. P., Sigurdsson, S. T. & Ferre-D'Amare, A. R. Transition state stabilization by a catalytic RNA. Science298, 1421–1424 (2002). ArticleCASPubMedGoogle Scholar
- Stahley, M. R. & Strobel, S. A. Structural evidence for a two-metal-ion mechanism of group I intron splicing. Science309, 1587–1590 (2005). ArticleCASPubMedGoogle Scholar
- Torres-Larios, A., Swinger, K. K., Krasilnikov, A. S., Pan, T. & Mondragon, A. Crystal structure of the RNA component of bacterial ribonuclease P. Nature437, 584–587 (2005). ArticleCASPubMedGoogle Scholar
- Westhof, E., Masquida, B. & Jaeger, L. RNA tectonics: towards RNA design. Fold. Des.1, R78–88 (1996). ArticleCASPubMedGoogle Scholar
- Lescoute, A. & Westhof, E. Topology of three-way junctions in folded RNAs. RNA12, 83–93 (2006). ArticleCASPubMedPubMed CentralGoogle Scholar
- De la Pena, M., Gago, S. & Flores, R. Peripheral regions of natural hammerhead ribozymes greatly increase their self-cleavage activity. EMBO J.22, 5561–5570 (2003). ArticleCASPubMedPubMed CentralGoogle Scholar
- Khvorova, A., Lescoute, A., Westhof, E. & Jayasena, S. D. Sequence elements outside the hammerhead ribozyme catalytic core enable intracellular activity. Nature Struct. Biol.10, 708–712 (2003). ArticleCASPubMedGoogle Scholar
- Krasilnikov, A. S., Yang, X., Pan, T. & Mondragon, A. Crystal structure of the specificity domain of ribonuclease P. Nature421, 760–764 (2003). ArticleCASPubMedGoogle Scholar
- Waldsich, C. & Pyle, A. M. A folding control element for tertiary collapse of a group II intron ribozyme. Nature Struct. Mol. Biol.14, 37–44 (2007). ArticleCASGoogle Scholar
- Nudler, E. Flipping riboswitches. Cell126, 19–22 (2006). ArticleCASPubMedGoogle Scholar
- Wickiser, J. K., Winkler, W. C., Breaker, R. R. & Crothers, D. M. The speed of RNA transcription and metabolite binding kinetics operate an FMN riboswitch. Mol. Cell18, 49–60 (2005). ArticleCASPubMedGoogle Scholar
- Wickiser, J. K., Cheah, M. T., Breaker, R. R. & Crothers, D. M. The kinetics of ligand binding by an adenine-sensing riboswitch. Biochemistry44, 13404–13414 (2005). ArticleCASPubMedGoogle Scholar
- Semrad, K. & Schroeder, R. A ribosomal function is necessary for efficient splicing of the T4 phage thymidylate synthase intron in vivo. Genes Dev.12, 1327–1337 (1998). ArticleCASPubMedPubMed CentralGoogle Scholar
- Ferat, J. L., Le Gouar, M. & Michel, F. A group II intron has invaded the genus Azotobacter and is inserted within the termination codon of the essential groEL gene. Mol. Microbiol.49, 1407–1423 (2003). ArticleCASPubMedGoogle Scholar
- Jenkins, K. P., Hong, L. & Hallick, R. B. Alternative splicing of the Euglena gracilis chloroplast roaA transcript. RNA1, 624–633 (1995). CASPubMedPubMed CentralGoogle Scholar
- Vogel, J. & Hess, W. R. Complete 5′ and 3′ end maturation of group II intron-containing tRNA precursors. RNA7, 285–292 (2001). ArticleCASPubMedPubMed CentralGoogle Scholar
- Przybilski, R. et al. Functional hammerhead ribozymes naturally encoded in the genome of Arabidopsis thaliana. Plant Cell17, 1877–1885 (2005). ArticleCASPubMedPubMed CentralGoogle Scholar
- Ferbeyre, G., Bourdeau, V., Pageau, M., Miramontes, P. & Cedergren, R. Distribution of hammerhead and hammerhead-like RNA motifs through the GenBank. Genome Res.10, 1011–1019 (2000). ArticleCASPubMedPubMed CentralGoogle Scholar
- Graf, S., Przybilski, R., Steger, G. & Hammann, C. A database search for hammerhead ribozyme motifs. Biochem. Soc. Trans.33, 477–478 (2005). ArticleCASPubMedGoogle Scholar
- Gill, T., Cai, T., Aulds, J., Wierzbicki, S. & Schmitt, M. E. RNase MRP cleaves the CLB2 mRNA to promote cell cycle progression: novel method of mRNA degradation. Mol. Cell. Biol.24, 945–953 (2004). ArticleCASPubMedPubMed CentralGoogle Scholar
- Sudarsan, N. et al. Tandem riboswitch architectures exhibit complex gene control functions. Science314, 300–304 (2006). ArticleCASPubMedGoogle Scholar
- Rodionov, D. A., Dubchak, I., Arkin, A., Alm, E. & Gelfand, M. S. Reconstruction of regulatory and metabolic pathways in metal-reducing δ-proteobacteria. Genome Biol.5, R90 (2004). ArticlePubMedPubMed CentralGoogle Scholar
- Putzer, H., Gendron, N. & Grunberg-Manago, M. Co-ordinate expression of the two threonyl-tRNA synthetase genes in Bacillus subtilis: control by transcriptional antitermination involving a conserved regulatory sequence. EMBO J.11, 3117–3127 (1992). ArticleCASPubMedPubMed CentralGoogle Scholar
- Lease, R. A. & Belfort, M. A trans-acting RNA as a control switch in Escherichia coli: DsrA modulates function by forming alternative structures. Proc. Natl Acad. Sci. USA97, 9919–9924 (2000). ArticleCASPubMedPubMed CentralGoogle Scholar
- Sudarsan, N., Barrick, J. E. & Breaker, R. R. Metabolite-binding RNA domains are present in the genes of eukaryotes. RNA9, 644–647 (2003). ArticleCASPubMedPubMed CentralGoogle Scholar
- Griffiths-Jones, S. et al. Rfam: annotating non-coding RNAs in complete genomes. Nucleic Acids Res.33, D121–D124 (2005). ArticleCASPubMedGoogle Scholar
- Borsuk, P. et al. L-arginine influences the structure and function of arginase mRNA in Aspergillus nidulans. Biol. Chem.388, 135–144 (2007). ArticleCASPubMedGoogle Scholar
- Hertel, K. J., Peracchi, A., Uhlenbeck, O. C. & Herschlag, D. Use of intrinsic binding energy for catalysis by an RNA enzyme. Proc. Natl Acad. Sci. USA94, 8497–8502 (1997). ArticleCASPubMedPubMed CentralGoogle Scholar
- Tanner, N. K. et al. A three-dimensional model of hepatitis δ virus ribozyme based on biochemical and mutational analyses. Curr. Biol.4, 488–498 (1994). ArticleCASPubMedGoogle Scholar
- Canny, M. D. et al. Fast cleavage kinetics of a natural hammerhead ribozyme. J. Amer. Chem. Soc.126, 10848–10849 (2004). ArticleCASGoogle Scholar
- Richards, F. M. & Wyckoff, H. W. in The Enzymes (ed. Boyer, P. D.) 647–806 (Academic, New York, 1971). Google Scholar
- Nahas, M. K. et al. Observation of internal cleavage and ligation reactions of a ribozyme. Nature Struct. Mol. Biol.11, 1107–1113 (2004). ArticleCASGoogle Scholar
- Rodionov, D. A., Vitreschak, A. G., Mironov, A. A. & Gelfand, M. S. Comparative genomics of the methionine metabolism in Gram-positive bacteria: a variety of regulatory systems. Nucleic Acids Res.32, 3340–3353 (2004). ArticleCASPubMedPubMed CentralGoogle Scholar
- Salehi-Ashtiani, K. & Szostak, J. W. In vitro evolution suggests multiple origins for the hammerhead ribozyme. Nature414, 82–84 (2001). ArticleCASPubMedGoogle Scholar
- Koonin, E. V. The origin of introns and their role in eukaryogenesis: a compromise solution to the introns-early versus introns-late debate? Biol. Direct1, 22 (2006). ArticleCASPubMedPubMed CentralGoogle Scholar
- White, H. B. 3rd. Coenzymes as fossils of an earlier metabolic state. J. Mol. Evol.7, 101–104 (1976). ArticleCASPubMedGoogle Scholar
- Hammann, C. & Westhof, E. Searching genomes for ribozymes and riboswitches. Genome Biol.8, 210 (2007). ArticleCASPubMedPubMed CentralGoogle Scholar
- Noeske, J. et al. An intermolecular base triple as the basis of ligand specificity and affinity in the guanine- and adenine-sensing riboswitch RNAs. Proc. Natl Acad. Sci. USA102, 1372–1377 (2005). ArticleCASPubMedPubMed CentralGoogle Scholar
- Serganov, A. et al. Structural basis for Diels–Alder ribozyme-catalyzed carbon–carbon bond formation. Nature Struct. Mol. Biol.12, 218–224 (2005). ArticleCASGoogle Scholar
- Ke, A., Ding, F., Batchelor, J. D. & Doudna, J. A. Structural roles of monovalent cations in the HDV ribozyme. Structure15, 281–287 (2007). ArticleCASPubMedGoogle Scholar
- Beattie, T. L., Olive, J. E. & Collins, R. A. A secondary structure model for the self-cleaving region of Neurospora VS RNA. Proc. Natl Acad. Sci. USA92, 4686–4690 (1995). ArticleCASPubMedPubMed CentralGoogle Scholar
- Torres-Larios, A., Swinger, K. K., Pan, T. & Mondragon, A. Structure of ribonuclease P — a universal ribozyme. Curr. Opin. Struct. Biol.16, 327–335 (2006). ArticleCASPubMedGoogle Scholar
- Woodson, S. A. Structure and assembly of group I introns. Curr. Opin. Struct. Biol.15, 324–330 (2005). ArticleCASPubMedGoogle Scholar
- Rolle, K., Zywicki, M., Wyszko, E., Barciszewska, M. Z. & Barciszewski, J. Evaluation of the dynamic structure of DsrA RNA from E. coli and its functional consequences. J. Biochem. (Tokyo)139, 431–438 (2006). ArticleCASGoogle Scholar
- Brown, L. & Elliott, T. Mutations that increase expression of the rpoS gene and decrease its dependence on hfq function in Salmonella typhimurium. J. Bacteriol.179, 656–662 (1997). ArticleCASPubMedPubMed CentralGoogle Scholar
- Masse, E., Escorcia, F. E. & Gottesman, S. Coupled degradation of a small regulatory RNA and its mRNA targets in Escherichia coli. Genes Dev.17, 2374–2383 (2003). ArticleCASPubMedPubMed CentralGoogle Scholar
- Yousef, M. R., Grundy, F. J. & Henkin, T. M. Structural transitions induced by the interaction between tRNA Gly and the Bacillus subtilis glyQS T box leader RNA. J. Mol. Biol.349, 273–287 (2005). ArticleCASPubMedGoogle Scholar
- Yousef, M. R., Grundy, F. J. & Henkin, T. M. tRNA requirements for glyQS antitermination: a new twist on tRNA. RNA9, 1148–1156 (2003). ArticleCASPubMedPubMed CentralGoogle Scholar
- Altuvia, S. & Wagner, E. G. H. Switching on and off with RNA. Proc. Natl Acad. Sci. USA97, 9824–9826 (2000). ArticleCASPubMedPubMed CentralGoogle Scholar
Acknowledgements
This work was supported by the US National Institutes of Health grant GM073618.